All published articles of this journal are available on ScienceDirect.
Microalgae Production: A Sustainable Alternative for a Low-carbon Economy Transition
Abstract
The production of microalgae on a commercial scale began in the 1970s. From this time until today it has consolidated itself as an alternative for human consumption and animal feed, mainly through aquaculture (carcinoculture, oyster farming, and fish farming).
Currently, most of the micro-algal biomass that has been produced in photoautotrophic systems for human consumption comes from four main genera (Chlorella, Arthrospira, Dunaliella, and Haematococcus). Recent advances allowed Nannochloropsis and Euglena cultivation in open ponds for feed and fuels.
Although the initiatives mentioned represent the success of the scale-up for microalgae production, there are challenges to be overcome for the use of the vast set of existing microalgae species.
The promising future of the industry involved in large scale production of microalgae is supported by its characteristic that is clearly sustainable from an ecological point of view and in the transition proposal to a low carbon economy that has been intensified in response to the effects caused by the progressive release of CO2 in the atmosphere.
Innovative applications from microalgae biotechnology are being developed every year. In this context, there have been several research and development initiatives over the past decade aimed at obtaining advanced fuels making full use of micro-algal biomass.
1. INTRODUCTION
Virtually all active ecosystems on the planet survive according to the so-called sustainability principles that, under certain environmental conditions, provide for the survival of communities in different ecosystems and their next generations over ecological time.
The economic development carried out over the last century took place in response to the new needs of today's societies, achieving success and providing food, vaccines, amenities, and comfort, extending human longevity in a way never seen before.
However, perhaps due to the little initial scientific knowledge about ecology, human activities, including human activities such as agriculture, trade and industrial production were conducted most of the time in spite of the four principles of sustainability described below, generating environmental degradation to a point also never seen by humanity [1, 2].
Terrestrial or aquatic ecosystems generally use a renewable energy source such as sunlight, capable of sustaining primary production stocks without interruption due to resource depletion.
The cycling of matter is essential for chemical compounds to be available in adequate quantity and proportion so that fundamental biological processes such as photosynthesis, respiration, and reproduction are not obliterated or impaired [3].
The conservation of biodiversity present in terrestrial or aquatic ecosystems is important in order not to cause population imbalances capable of affecting future generations with the uncontrolled proliferation of parasites and predators [4, 5].
Finally, the fourth principle advocates the non-use of energy and material resources beyond what is necessary (overconsumption) for the maintenance of individuals present in the communities of terrestrial or aquatic ecosystems, causing a shortage or absence of resources for the next generations.
The cultivation of microalgae on a larger scale is an activity that has been established and improved since the 1950s to the present day [6], has a fully sustainable action status from the scientific point of view, and according to the results of its commercial ramifications [7]. The various production initiatives have developed over time to serve different purposes such as organic sewage treatment, production of nutraceuticals, cosmetics, food, biofuels, biofertilizers, and fine chemicals [8, 9].
However, there may be an association in the microalgae biomass production processes (upstream) that can be carried out in different scenarios using organic sewage from large cities or effluents from oil and gas refineries with the subsequent conversion of the biomass obtained into biofuels (downstream) although the latter does not yet have fully defined routes being the subject of many R&D activities today [10].
Microalgae grown in environments outside the laboratory use a renewable and low-cost energy source (sunlight) while promoting the biofixation of CO2 and other nutrients containing P, N, and Mg intensively due to their high productivity, contributing to the cycling of matter more efficiently than current agriculture due to losses associated with volatilization and leaching of compounds present in chemical fertilizers such as urea and superphosphates [11].
Microalgae cultivation systems do not require fertile soils or areas with high biodiversity in the same way as other activities belonging to agribusiness and can be established in a complementary way to pre-existing agricultural activities using brackish water, water containing surplus agricultural fertilizers, or industrial effluent water (e.g., water from an oil refinery) thus preserving biodiversity [12].
The cultivation activities are included in a renewable energy matrix that can be coupled with wind or solar energy for moving crops; besides it uses nutrients with high efficiency, converting much of the carbon, phosphorus, and nitrogen added to the medium avoiding the waste of natural resources related to overconsumption [13, 14].
The microalgae production sites active on the planet reflect, according to the above characteristics, a modern sustainability emblem with great potential to leverage local and regional development in several countries (Israel, USA, Chile, Japan, Brazil, China, Australia) collaborating for the preservation of the environment as well as the generation of economic and social value.
This review addresses some important steps and challenges for producing microalgae on a larger scale and presents some recent possibilities for sustainable applications in connection with agricultural and industrial activities.
2. CURRENT CHALLENGES FOR PHOTOAUTOTROPHIC SYSTEMS SCALE-UP
Although there are hundreds of species of marine or freshwater algae being grown in the laboratory for the most diverse purposes (e.g., taxonomic classification, collection of strains, biochemical composition) [15], there are few genera of microalgae being produced on a larger scale, with an estimated total annual production of 20,000 tons (ash-free dry weight), (ash-free dry weight) , still very small when compared to traditional agricultural production of vegetables such as soybeans, corn, and rice [16, 17].
Over the last 50 years, microalgae of the genera Chlorella, Dunaliella, Arthrospira, Haematococcus have stood out for human consumption and, on a smaller scale, Nannochloropsis, with the potential to expand its production in the near future (Fig. 1).

Haematococcus pluvialis enriched in astanxanthin (1% by mass) is marketed as a nutraceutical product due to its antioxidant properties that benefit the strengthening of the human immune system. Its detailed biochemical composition was determined in a technical review published by the company Cyanotech [18].
Nannochloropsis was also used in R&D activities to obtain advanced fuels by the companies Exxon Mobil and Synthetic Genomics, having obtained expressive results for lipid productivity [19] in the USA (Fig. 2).

Aurora Biofuels cultivated Nannochloropsis in western mainland Australia to obtain biodiesel, EPAs, and animal feed. With the same objective, microalgae of the Staurosira and Desmodesmus genera were researched and used in Hawaii by the company Cellana - joint venture formed by the Companies Shell and HR BioPetroleum, in the scale-up process [20].
Initiatives for the production of ethanol from microalgae were also carried out, having highlighted the North American company Algenol that developed closed photobioreactors with CO2 injection in the culture medium containing genetically modified cyanobacteria capable of producing and exporting ethanol to the liquid medium, thus facilitating its recovery [21].
Euglena cultivation for fuels and feed has been developed by the Japanese company Euglena co. A pilot-scale plant started activities in 2019 at La Loma, Colombia. Euglena Co., along with Itochu corp., claim a perspective to scale up in Japan (Ishigaka Island) to produce 160 tons of Euglena biomass for jet fuel until 2025.
Through the enterprises and projects mentioned, scale increases were made aiming at the commercial production of microalgae biomass or compounds of interest present in biomass.
However, there is one characteristic inherent to all: its concrete insertion in the principles of sustainability mentioned at the beginning of this article.
They participate in the matter in the matter cycle by having CO2 as their main chemical insum, are based on the capture and supply of renewable energy, collaborate to maintain biodiversity, and can be carried out without overconsumption of available natural resources.
Some ventures were successful from a scientific point of view, reaching the desired economy, while others did not achieve the success initially imagined.
Often the methods of cultivation, harvesting, and conversion of microalgae biomass in the laboratory have excellent yields under extremely controlled conditions without high investment or operational costs for using minimal amounts of reagents, energy, and small equipment.
From the initial moment when the scale-up begins to be projected and made, the final economic result changes. Every small decrease obtained for investment or operating costs is reflected in the economic improvement of the various products to be obtained (e.g., agricultural fertilizers, biofuels, nutraceuticals).
The challenges that arise for obtaining biomass on a larger scale are spread over several stages of the cultivation process (Fig. 3), starting with the selection of native strains (Fig. 4) able to resist well to abiotic factors and biotic factors, with emphasis on the control of biological contaminations in open systems, ensuring cultivation stability until the harvesting operation.
The existing physicochemical and biological parameters exert influence (positive or negative) on the stability, productivity achieved as well as on the possible accumulation of target molecules in the produced biomass.
The generation of clean, high-quality inoculum containing healthy cells is essential for the success of the increase in the scale of crops.
The quality control of the inoculum is not a trivial task. It requires specialized personnel as well as expeditious and accurate analytical methodologies. In the initial phase of the culture preparation, the use of closed photobioreactors (Fig. 5) assumes relevant relevant importance to avoid the presence of possible interferences (chemical or biological) that can generate negative reflexes in the production process [22].
Stability is a fundamental condition for obtaining high productivity (above 20 g/m2/day) remaining as a scientific challenge as it does not dispense specialized knowledge of biochemistry and microbiology that can be applied to generate “crop protection” avoiding the occurrence of crashes by zooplankton predators (e.g., rotifers, fungi, or protozoa) or situations that involve competition for lighting and nutrients by invading microalgae.
Research activities on the chemical composition and function of allelopathic compounds (info-chemicals) present in the culture medium can help create mechanisms to assist in the control of biological contamination, reinforcing the resilience of crops in outdoor environments [23].
The target molecules control in the microalgae of interest is another scientific challenge that can be overcome only with the fine adjustment of biotic and abiotic parameters that may include salinity, pH, as well as the concentrations of dissolved oxygen, nitrogen, phosphorus, iron, and magnesium that are found in the culture medium [24].
The harvesting of biomass from crops is always a sensitive and particular step, depending on the species of microalgae cultivated and its final use (e.g., nutraceutical, cosmetic, food).
Direct filtration can be used to collect filamentous microalgae in a simple way on a smaller or larger scale, as in obtaining Arthrospira platensis wet biomass.
Microalgae that remain in the medium as individual cells and of reduced size (diameter less than 10 µm) can be harvested using other technologies, among which centrifugation, flocculation, flotation, or microfiltration stand out.
To obtain products with low added value, such as biofuels, harvesting through flocculation or autoflocculation is adequate due to the low energy cost spent [25]. The use of centrifugation or microfiltration can compromise the economy and is normally used to obtain nutraceuticals or fine chemicals due to the high investment and operational costs required.

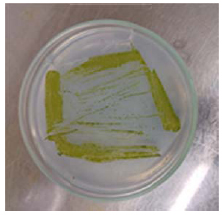

3. HETEROTROPHIC SYSTEMS – ANOTHER PATHWAY FOR MICROALGAL BIOMASS PRODUCTION
Some species of microalgae (e.g., Schizotrychium sp., Chlorella vulgaris, Chryptecodinium conii, and Tetraselmis gracilis) can grow rapidly in the absence of light using organic molecules (e.g., glucose, acetic acid, glycerin) as a substrate for biosynthesis of other carbohydrates (e.g., starch), lipids and proteins.
Schizochytrium sp. and Chryptecodinium conii have been successfully cultivated since the 1990s in heterotrophic systems by Martek Biosciences for the production of Polyunsaturated Fatty Acids (PUFAs) for early childhood feeding.
Heterotrophic crops are advantageous compared to photoautotrophic crops from the point of view of productivity. In this cultivation approach some species of microalgae can reach a high biomass concentration (80 g/l) at the end of a cultivation cycle (e.g., five days).
The fermenters used allow better control of the cultivation parameters , while closed SYSTEMS also represent an advantage for obtaining final biomass with high concentrations of target molecules (e.g., the total lipid content of 30% in Chlorella vulgaris).
However, the high yields obtained (e.g., 16g/l/day) depend on a number of factors which in turn prove to be of little advantage in relation to photoautotrophic cultivation systems. An important factor is related to the high costs of acquiring and maintaining pharmaceutical-grade fermenters that require sterilization and the addition of antibiotics to prevent the proliferation of bacteria that can compete advantageously for the added substrate with microalgae (Fig. 6).
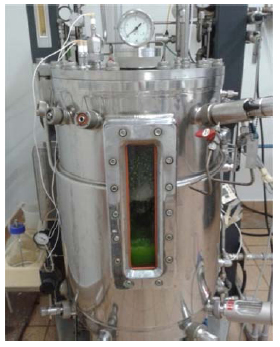
High added value products (e.g., paramylon, PUFAs), however, justify the use of heterotrophic crops despite their high energy cost associated with intensive aeration and mixing.
Over the last decade, the North American company Solazyme has produced advanced fuels (biodiesel and green diesel) from genetically modified microalgae and under heterotrophic growth, claiming to have reached a lipid content of 80% in the biomass produced. A joint venture was established between Solazyme and Bunge for the production of special drilling fluids in São Paulo, Brazil, which did not obtain the expected commercial success, evidencing the difficulties imposed by the scale-up process.
Euglena gracilis has been grown in fermenters to produce paramylon polysaccharide [26], which is marketed as anti-carcinogen in the nutraceutical market.
Heterotrophic systems consume carbon from elaborate molecules and not in the form of CO2, which makes it difficult to insert them into transition processes to a low-carbon economy to increase sustainability.
CO2-rich effluents from the oil and gas industries, steel mills, sugarcane, and cement plants, among others, could not be used directly as insum for heterotrophic microalgae production systems.
4. AN INTEGRATED APPROACH WITH THE OIL AND GAS INDUSTRY
The supply of CO2 for microalgae cultivation systems can be carried out from various industrial effluents. The mixture of gases generated by the combustion of coal or natural gas in thermoelectric plants can be used directly after cooling and washing. Another possibility is the use of CO2 from the initial processing of oil and natural gas produced.
In addition to the use of the associated CO2 as a nutrient for microalgae, another input derived from the oil and gas production is the produced water whose composition depends on each specific reservoir.
Genetically resistant native microalgae achieved excellent growth in open ponds on the pilot scale using pure petroleum-produced water with added nutrients, a project conducted by Petrobras and the Federal University of Rio Grande do Norte (Fig. 7). At the end of the cultivation cycle, polluting compounds initially (e.g., ammonia) present in the water were assimilated by the microalgae.

The biomass of Scenedesmus produced can be subjected to the cold extraction of its oil content and its conversion to advanced fuels, with the remaining biomass being used as organic fertilizer for agriculture with excellent results. In this way, there is total integration with agricultural activities without any residual stuff at the end of the process (Fig. 8).
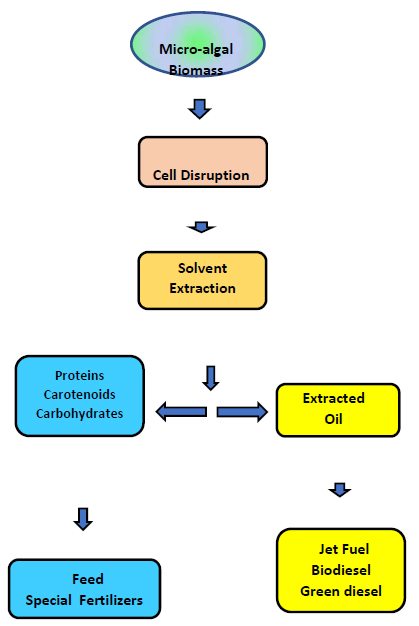
Green microalgae have a good perspective to produce high-value carotenoids (e.g., Lutein) and lipids in photoautotrophic cultivation systems. Some microalgae genera (Scenedesmus, Nannochloropsis, Chlorella, and Chlorococcum) can be fully used following the development of a biorefinery concept [27-29].
5. OFFSHORE CROPS: A NEW SCIENTIFIC AND TECHNOLOGICAL FRONTIER
Currently, several companies (e.g., Equinor, Shell, Chevron, and Petrobras) have part of their reserves in the offshore region, needing to develop new technologies to mitigate CO2 emissions associated with the production of oil and gas in the marine units.
The cultivation of microalgae or macroalgae in the offshore environment is still in an embryonic stage, belonging to a new technological frontier that unites oceanography and biotechnology [30]. However, it has great potential for biofixation of CO2 in oceanic regions where oil exploration and production platforms are located.
Some R&D initiatives have been carried out for the cultivation of microalgae in the USA [31] and for the small-scale macroalgae cultivation in Norway [32] without CO2 injection during the growth process.
Fixed production platforms in shallow water (20 to 50 m deep) need to be decommissioned after depleting oil and gas reserves. These maritime units (Fig. 9) can be used as oceanographic bases for carrying out experiments on a pilot-scale aiming at the growth of microalgae or macroalgae with a regulated supply of CO2 in the seawater.
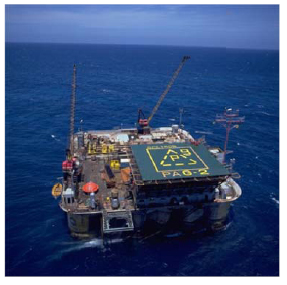
According to the productivity performance and the conversion rates of CO2 into algae biomass obtained under oceanic conditions, an eventual scale-up can be better evaluated under an economic approach.
CONCLUSION
The production of microalgae, which has been carried out and improved over the last fifty years, is a fully sustainable activity from an ecological point of view and can become economically viable depending on the success obtained along the success achieved during the outdoor crops scale-up.
The scale-up processes related to the cultivation and production of biomass, in turn, offer challenges from a scientific and technological point of view in order to achieve conditions of stability and high productivity.
Microalgae crops with CO2 capture to produce advanced fuels (biodiesel, green diesel, and jet fuel) are at the technological edge of bioenergy and have great potential for use in the transition to a low-carbon economy.
New products obtained from microalgae have recently been developed with a special emphasis on fuels, nutraceuticals, fertilizers, and fine chemicals, following the concept of full use of biomass through biorefineries.
Microalgae and macroalgae cultivation in offshore environments still have few initiatives in terms of research and development and can collaborate in the processes of capturing CO2 from oil and gas production activities in the marine environment.
The development of new strains using genetic engineering to obtain target molecules has been collaborating positively to obtain economics in microalgae crops aimed at commercial enterprises.
CONSENT FOR PUBLICATION
Not applicable.
FUNDING
None.
CONFLICT OF INTEREST
The authors declare no conflict of interest, financial or otherwise.
ACKNOWLEDGEMENTS
Declared none.